
Cathodoluminescence g(2) Autocorrelation
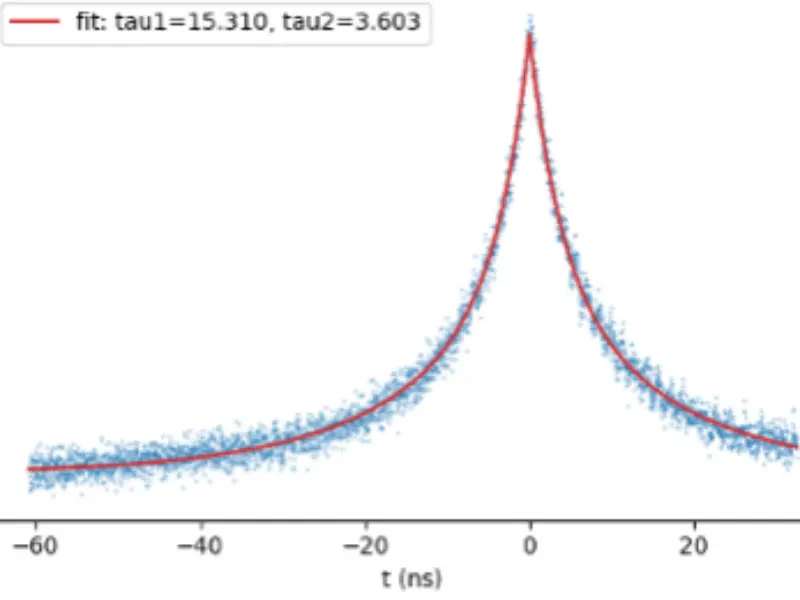
Introduction
Time-correlation of light intensity, also known as g2 autocorrelation, is a fundamental property of a light source. The g2 notation denotes the probability of detecting two photons spaced by a time delay from a light source. Single-photon emitters, among other non-trivial light sources, are unequivocally characterized by their g2 curves. Autocorrelation measurements are thus useful in optical quantum computing and quantum communication research. In the context of cathodoluminescence (CL) specifically, g2 can also reveal luminescence lifetimes in a sample. Recent research has demonstrated that the technique is generally applicable in a Time-Resolved cathodoluminescence (TR-CL) measurement scheme.
Principles
At the fundamental level, random fluctuations occur in the intensity of any light source. In practical terms, intensity measurements on very short time scales will reveal fluctuations in a light source even if its intensity is constant when observed on longer time scales. In a stream of individually-detected photons, intensity fluctuations are expressed as the conditional probability of detecting a photon at a time delay after a first detection has occurred. The nature of these fluctuations allows to classify light sources in three categories: thermal light, coherent light and sub-Poissonian light. The latter is mostly encountered as single-photon emission.
Examples of the three radiation categories are illustrated below. Thermal light, such as radiation emitted from the sun, LEDs, incandescent and fluorescent lightbulbs, exhibits photon bunching. Photons are more likely to reach the detection setup in groups. Coherent light, encountered in laser sources, is completely uncorrelated. Photons reach the detector at random times independent of previous detection events. Finally, single photon emitters such as quantum dots, individual fluorescent molecules and color centers in dielectric materials observe a stochastic pause time between the emission of successive photons. Their distribution in time is more evenly spaced than for coherent and thermal light.
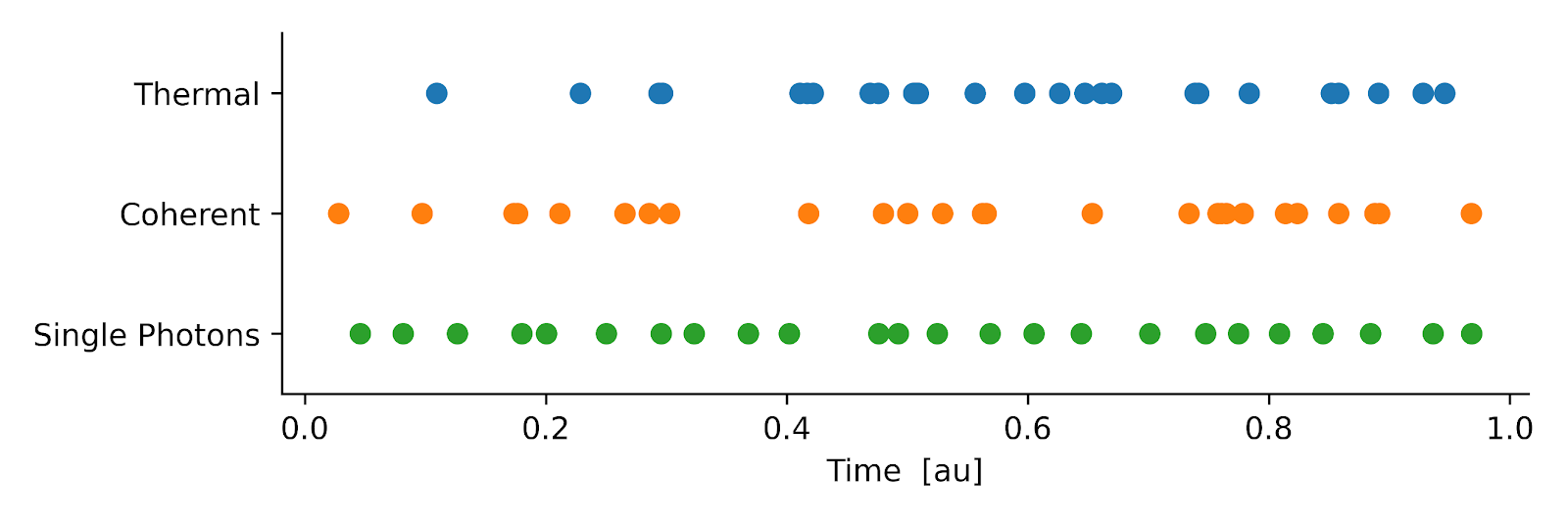
These behaviors are quantitatively represented using histograms of the time delay between arrival times of photon pairs:
g2=〈ItI(t+τ)〉〈It〉2
For thermal light, the bunching behavior is shown as a positive correlation on the histogram, while for coherent light the histogram remains flat. For sub-Poissonian light, the histogram shows negative correlation, and single photon emission is proved when anti-correlation crosses the limit of 0.5 at zero time-delay.
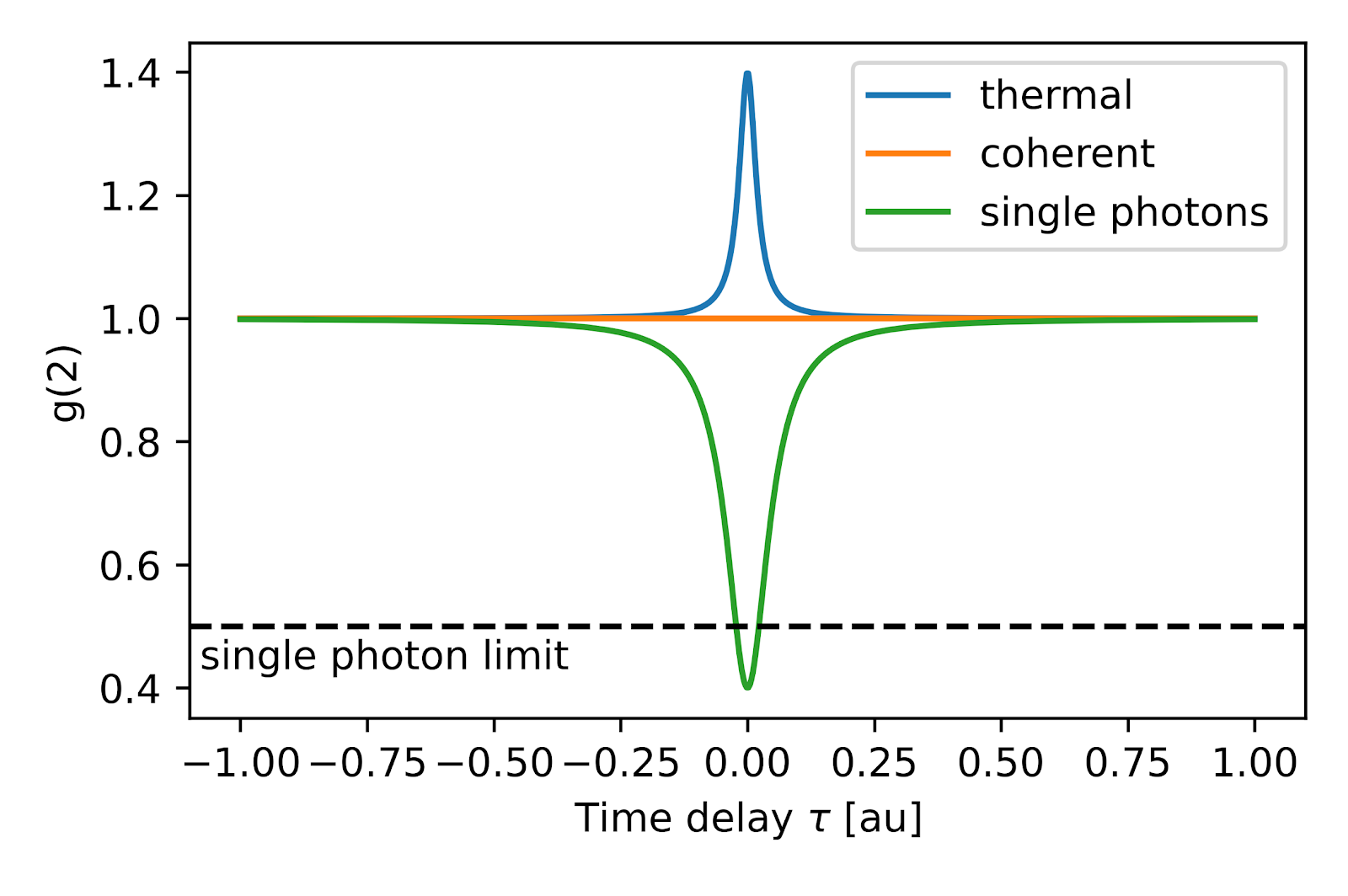
Recently, scientists demonstrated that particular ways of generating light emission from a sample can induce non-trivial modifications to the g(2) curve. Specifically, in cathodoluminescence a single energetic electron from the beam can trigger emission of hundreds of photons in the moment after its interaction with the sample. This induces a large bunching peak in the CL g(2) histogram, directly linked to the luminescence lifetime. As long as the probe current is low enough, primary electrons are sufficiently spaced in time and the g(2) curve does not depend on their precise arrival time, and luminescence lifetimes can be measured without using a pulsed electron beam.
This novel way of performing time-resolved measurements is now used in several labs around the world and is gaining popularity in the CL community. As it does not require a pulsed electron beam system, it is a very cost-effective solution to performing TR-CL measurements. g2 autocorrelation also remains the gold standard for proving single photon emission, and its implementation in CL instruments offers the added benefit of allowing highly localized excitation distribution available with focused electron beams.
Instrumentation
Timing streams of photons requires both single photon and time-resolved detection. In practice, timing cannot be achieved with a single detector. Single photon detectors such as Single-Photon Avalanche Detectors (SPADs), Photomultiplier Tubes (PMTs) and Hybrid Photomultiplier Detectors (HPDs) exhibit dead times necessary to amplify the signal after capture of a single photon. Even short dead times, in the range of nanoseconds, are too long for most applications. Historically, this problem has been solved in an apparatus nowadays called the Hanbury Brown and Twiss (HBT) interferometer.
A diagram of the setup is shown below. The signal is partitioned on two detectors connected to time-correlation electronics. The correlation electronics works like a chronometer, where a first detection event starts the timer and a second event stops it. By accumulating enough events, the g(2) histogram is built directly. To a large extent, HBT setups conserve the same functioning to this day. Modern correlation electronics allow to record streams of photons in individual channels directly, and the histograms are numerically computed from this information. Some manufacturers use the term “timing electronics” for this reason.
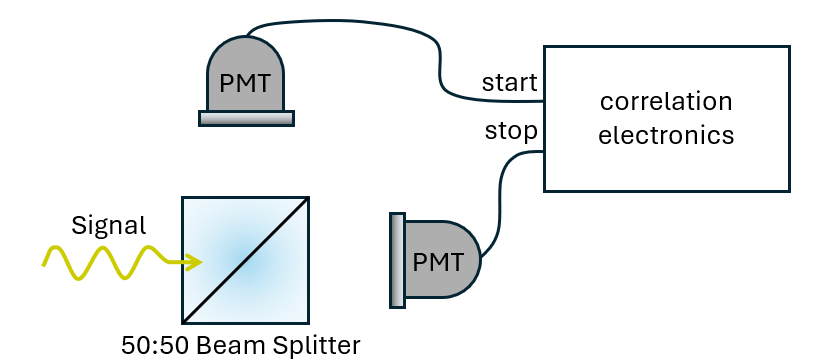
Modern timing electronics also allow multiplexing of the channels, higher dynamic range and implement separate synchronization channels. On the Allalin platform, the idea behind the HBT setup implementation is to create a modular detection system capable of realizing g(2) autocorrelation, TR-CL, TR-PL and more advanced experiments in the same setup. It can implement all state-of-the-art single-photon detectors in the setup, offer both fiber and free space coupling for spectral selection through filters and monochromators.
Example results

Figure 4 Effect ofprobe current on g2 bunching in the luminescence of GaN. Left: G2 curves fittedwith bi-exponential decays for various probe currents. Right: g2 bunching peakheight and lifetime vs probe current. Bunching height shows exponentialdependency on probe current, while lifetime remains constant.
Benefits and Further Reading
- A founding article on g(2) measurements using cathodoluminescence, and its application on characterization of single photon emitters. https://journals.aps.org/prl/abstract/10.1103/PhysRevLett.110.153604
- Seminal paper on the use of CL-g(2) as a technique to perform lifetime measurements using continuous electron beams https://journals.aps.org/prl/abstract/10.1103/PhysRevLett.114.197401
Latest Scientific Publications
Explore our high-resolution cathodoluminescence tools designed for superior materials characterization.